Although wetlands make up a small percentage of total land area in the United States, they store a disproportionate amount of carbon largely due to their unique hydrology. Here, a basic summary of how carbon is stored and moves through these wetlands, and how climate change and management may interact with site conditions to alter wetland carbon cycling, is provided.
Where is Carbon Stored in Wetland Ecosystems?
The amount of carbon stored in a wetland is a function of both the rate at which carbon is captured and secured in the ecosystem (carbon sequestration) and how long the carbon persists in the system. Like upland ecosystems, carbon is stored in different pools in wetlands. Live aboveground plant carbon and live belowground plant carbon refer to the amount of carbon stored in plant biomass aboveground and belowground, respectively, including macrophytes and algae. Given that plant biomass is approximately 50% carbon, biomass can be used as a proxy to estimate plant carbon. Once a plant dies, plant carbon is transferred to particulate organic carbon or dissolved organic carbon pools. These detrital carbon pools exist in both the water column and make up part of the soil carbon pool.
Carbon Storage
Carbon Sequestration
The rate at which carbon is captured and secured in the ecosystem (or soil).
The relative sizes of wetland carbon pools vary considerably. Compared to upland ecosystems, wetlands tend to store a greater percentage of total ecosystem carbon in soils due to both smaller plant carbon pools (particularly for non-forested wetlands) and larger soil carbon pools. The ratio between live aboveground versus belowground plant carbon is much more variable in wetlands compared to uplands systems, with some wetlands storing more plant carbon aboveground and others more belowground (Reddy et al. 2023). Soil carbon pools are larger in organic versus mineral soils. Compared to other regions of the continental United States, wetlands in the Eastern and Midwest region have the largest soil carbon pools due to the large quantities of carbon stored in deep soils (Nahik and Fennessy 2016).
How Does Carbon Cycle Through Wetland Ecosystems?
Carbon sequestration is the balance of how much new carbon enters a wetland versus how much carbon is lost via gaseous emissions (largely from organic matter decomposition) or leaching. In upland ecosystems, climate broadly dictates plant productivity and therefore the rate of new carbon inputs. In contrast, soil properties and hydrology are much stronger controls on wetland productivity (Schlesinger and Bernhardt 2020).

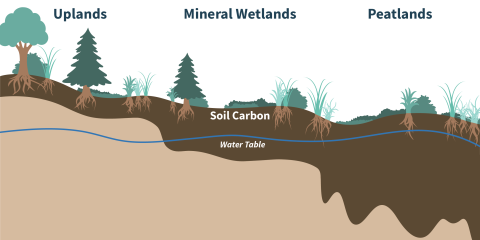
Rates of primary productivity are broadly similar between upland and wetland ecosystems, but organic matter decomposition is much slower in wetlands, leading to faster accumulation of soil carbon (Fig. 1). The unique hydrology of wetlands not only slows overall decomposition but also alters decomposition pathways and products. In upland ecosystems, organic matter decomposition is largely aerobic (i.e., carried out in the presence of oxygen) and produces carbon dioxide. However, higher water tables in wetlands create conditions with low or no oxygen, which drive anaerobic (i.e., carried out in the absence of oxygen) decomposition. One common product of anaerobic decomposition is methane, a more potent greenhouse gas compared to carbon dioxide. Methane can also be consumed by certain microbes. Therefore, net carbon emissions from wetlands are largely driven by the ratio of carbon dioxide to methane production (dictated by oxygen concentrations and therefore hydrology) and the relative production versus consumption of methane (Fig.2).
Differences in hydrology between peatlands and mineral wetlands result in peatlands storing vastly more carbon in soils compared to mineral wetlands. Peatlands generally have high and stable water tables throughout the growing season, leading to sustained anaerobic conditions and slow decomposition. By contrast, mineral wetlands can dry out during part of the growing season, enhancing aerobic decomposition and increasing carbon losses.
Various Effects on Wetland Carbon
The conditions that dictate the type (carbon dioxide versus methane) and rate of carbon emissions from wetlands change rapidly over space and time. As a result, wetland carbon cycling is highly variable and challenging to accurately quantify both within and across sites. Within one wetland, carbon emissions can vary by orders of magnitude both seasonally and across space at a given point in time. Spatially, soil carbon storage and sequestration is generally highest in the inner wetland and decreases towards the wetland edge and upland (Tangen and Bansal 2020). Land managers can consider the following site characteristics to better understand wetland carbon cycling at a site.
- Although variable, fens generally emit more methane than other wetland types (e.g., bogs) (Turetsky et al. 2014).
- Vegetation is an important control on wetland carbon cycling, but it is not possible to provide general predictions based on vegetation type. Still, grasses and sedges often enhance methane emissions given their capacity to transport methane from deep low-oxygen zones, through specialized plant tissues called aerenchyma, and into the atmosphere (Zhang et al. 2002, Turetsky et al. 2014). Through this process, methane gasses bypass oxygen-rich zones where methane would otherwise be converted to carbon dioxide.
- Plant tissue chemistry affects decomposition. Plant inputs that have lower lignin: cellulose ratios (i.e., higher ‘quality’ inputs) seem to result in greater methane production as well as faster overall decomposition (Duval and Radu 2018).
- Sites with high sulfate concentrations tend to have low methane emissions because sulfate suppresses methane production.
- Peatlands, and to a lesser degree fine-textured mineral soil wetlands, store the greatest amount of carbon due to their combined high capacity to store carbon and low rates of decomposition.
Carbon Dioxide and Methane
Wetlands are considered more sensitive to climate change than many upland ecosystems. Unfortunately, the direction (source vs. sink) and magnitude of climate change effects on wetland carbon cycling at a given site remains challenging to predict. Peatlands and fine-textured soils will generally experience the greatest absolute changes in carbon storage under climate change due to their high carbon storage (Tangen and Bansal 2020).
Wetland carbon cycling responses to climate change reflect the combination of direct effects of changes in temperature and precipitation along with indirect effects on the plant and soil microbial community. In general, warmer and wetter conditions favor methane over carbon dioxide production and are thus expected to stimulate the greatest increases in wetland carbon emissions. Drier conditions, predicted across much of the Midwest and Northeast, may dampen the effects of warming temperatures. When water table levels drop, oxygen availability (and therefore decomposition) increases. Although this can stimulate short-term increases in methane emissions (Knox et al. 2021), if water table levels remain low, methane emissions should decrease while carbon dioxide emissions increase. Altered hydrology may also indirectly affect wetland carbon cycling. Extreme precipitation events are expected to continue to increase with climate change, which will increase erosion and nutrient runoff in upland systems. This could increase external carbon inputs and wetland fertility, particularly for wetlands lower in the watershed.
Increases in fertility, particularly in formerly low-nutrient wetlands, will favor non-native, fast-growing invasive species. Invasive species may increase primary productivity (i.e., carbon sequestration) and plant-derived carbon storage over the short-term but could also increase methane emissions. For example, dominance of the nonnative and invasive cattail, Typha x glauca, has been related to higher methane emissions. More generally, plant productivity and methane emissions are positively related (Tangen et al. 2015). However, the relationship between plant productivity and overall wetland carbon storage is less predictable given the many factors that influence organic matter decomposition.
Changes in the plant community can affect organic matter decomposition through changes in the quantity and chemistry of organic matter inputs and effects on the microbial decomposer community. For example, carbon cycling in Sphagnum moss-dominated bogs may be particularly vulnerable to changes in plant species: bogs tend to have low methane emissions, which may be due to the unique effects of Sphagnum on organic matter decomposition (Bridgham et al. 2013). While predicting how plant community changes will affect wetland carbon is difficult, reduced native plant diversity increases wetland climate vulnerability and may result in carbon losses over longer time scales
Currently, North American wetlands are a carbon dioxide sink (sequestering more carbon dioxide than is released) but a source of methane to the atmosphere (Kolka et al. 2018). Although wetland methane production is a significant source of greenhouse gas emissions globally, wetland drainage increases overall emissions due to rapid losses of large soil carbon reservoirs. Given that large stores of soil carbon are protected from decomposition in wetlands, wetland drainage and conversion to other land uses presents the biggest threat to large and rapid greenhouse gas emissions from wetlands. Upon draining, large amounts of soil organic matter will be rapidly decomposed, releasing carbon into the atmosphere. In particular, peatland conversion to agriculture can result in large carbon losses and greenhouse gas emissions. Converting peatlands to agricultural land typically involves intensive drainage using tiles and fertilization, which stimulates high carbon dioxide and nitrous oxide (a greenhouse gas more potent than carbon gases) emissions, and resulting ditches can also be hot spots for methane emissions.
More moderate changes in wetland hydrology also affect wetland carbon cycling, albeit to a lesser extent versus land use change. Increasing connectivity of wetlands could buffer wetlands from short-term fluctuations in water table levels (which tends to stimulate carbon emissions) and may increase total inner wetland area (where carbon storage and sequestration tends to be highest).
Management of invasive plant species also affects long-term wetland carbon cycling, depending on plant species identity, the specific management action, and interacting site factors. Research on Typha x glauca suggests that harvesting and crushing treatments commonly create floating mats of live and dead biomass; while floating mats provide some benefits, they can also contribute to methane emissions (Johnson et al. 2021). Prescribed fires can be used for invasive species management in wetlands. Soil carbon losses from prescribed fire are often minimal in mineral wetlands, but significant soil carbon can be lost with fire in peatlands where fires can burn unabated for years. Overall, how invasive plant species management influences wetland carbon cycling warrants further research.
Creed, I.F.; Miller, J.; Aldred, D.; Adams, J.K.; Spitale, S.; Bourbonniere, R.A. 2013. Hydrologic profiling for greenhouse gas effluxes from natural grasslands in the prairie pothole region of Canada. Journal of Geophysical Research: Biogeosciences, 118: 680–697. doi.org/10.1002/jgrg.20050.
Duval, T.P.; Radu, D.D. 2018. Effect of temperature and soil organic matter quality on greenhouse-gas production from temperate poor and rich fen soils. Ecological Engineering, 114: 66–75. doi.org/10.1016/j.ecoleng.2017.05.011.
Johnson, O.F.; Panda, A.; Lishawa, S.C.; Lawrence, B.A. 2021. Repeated large-scale mechanical treatment of invasive Typha under increasing water levels promotes floating mat formation and wetland methane emissions. Science of The Total Environment, 790: 147920. doi.org/10.1016/j.scitotenv.2021.147920
Knox, S. H.; Bansal, S.; McNicol, G.; Schafer, K.; turtevant, C.; Ueyama, M.; Valach, A.C.; Baldocchi, D.; Delwiche, K.; Desai, A.R.; EEuskirchen, E.; Liu, J.; Lohila, A.; Malhotra, A.; Melling, L.; Riley, W.; Runkle, B.R.K.; Turner, J.; Vargas, R.; Zhu, Q.; Alto, T.; Fluet-Chouinard, E.; Goeckede, M.; Melton, J.R.; Sonnentag, O.; Vesala, T.; Ward, E.; Zhang, Z.; Feron, S.; Ouyang, Z.; Alekseychik, P.; Aurela, M.; Bohrer, G.; Campbell, D.I.; Chen, J.; Chu, H.; Dalmagro, H.J.; Goodrich, J.P.; Gottschalk, P.; Hirano, T.; Iwata, H.; Jurasinski, G.; Kang, M.; Koebsch, F.; Mammarella, I.; Nilsson, M.B.; Ono, K.; Peichl, M.; Peltola, O.; Ryu, Y.; Sachs, T.; Sakabe, A.; Sparks, J.P.; Tuittila, E.; Vourlitis, G.L.; Wong, G.X.; Windham-Myers, L.; Poulter, B.; Jackson, R.B. 2021. Identifying dominant environmental predictors of freshwater wetland methane fluxes across diurnal to seasonal time scales. Global Change Biology, 27: 3582–3604. doi.org/10.1111/gcb.15661.
Nahlik, A.M.; Fennessy, M.S. 2016. Carbon storage in US wetlands. Nature Communications, 7: 13835. doi.org/10.1038/ncomms13835
Reddy, K.R.; DeLaune, R.D.; Inglett P.W. 2023. Biogeochemistry of wetlands: science and applications (2nd edition). Boca Raton, CRC Press. doi.org/10.1201/9780429155833
Schlesinger, W.H.; Bernhardt, E. 2020. Biogeochemistry: an analysis of global change. 4th edition. New York, Elsevier/Academic Press. doi.org/10.1016/C2017-0-00311-7
Tangen, B.A.; Bansal, S. 2020. Soil organic carbon stocks and sequestration rates of inland, freshwater wetlands: Sources of variability and uncertainty. Science of The Total Environment, 749: 141444. doi.org/10.1016/j.scitotenv.2020.141444
Tangen, B.A.; Finocchiaro, R.G.; Gleason, R.A. 2015. Effects of land use on greenhouse gas fluxes and soil properties of wetland catchments in the Prairie Pothole Region of North America. Science of The Total Environment, 533: 391–409. doi.org/10.1016/j.scitotenv.2015.06.148
Turetsky, M.R.; Kotowska, A.; Bubier, J.; Dise, N.B.; Crill, P.; Hornibrook, E.R.C.; Minkkinen, K.; Moore, T.R.; MyersSmith, I.H.; Nykänen, H.; Olefeldt, D.; Rinne, J.; Saarnio, S.; Shurpali, N.; Tuittila, E.S.; Waddington, J.M.; White, J.R.; Wickland, K.P.; Wilmking, M. 2014. A synthesis of methane emissions from 71 northern, temperate, and subtropical wetlands. Global Change Biology, 20: 2183–2197. doi.org/10.1111/gcb.12580
Zhang, Y.; Li, C.; Trettin, C.C.; Li, H.; Sun, G. 2002. An integrated model of soil, hydrology, and vegetation for carbon dynamics in wetland ecosystems. Global Biogeochemical Cycles, 16: 9-1-9–17. doi.org/10.1029/2001GB001838
Suggested Citation of the Hub Publication
Keller, A.B. and Handler, S. 2024. Carbon in Non-Forested Wetlands of the Midwest and Eastern United States: A Primer. Technology Transfer. Houghton, MI: U.S. Department of Agriculture, Northern Forests Climate Hub. 6 p.
Acknowledgments
This is a product of the USDA Northern Forests Climate Hub and the Northern Institute of Applied Climate Science, a collaborative, multi-institutional partnership led by the USDA Forest Service. Funding was provided by the USDA Forest Service and The Nature Conservancy.